Seed Dormancy
Seed Dormancy Webpage 1 (this webpage)
What is seed dormancy and how is it related to germination? -
Definition of seed dormancy
A classification system for seed dormancy: The 'whole-seed view'
Evolution of seed structure and seed dormancy
Embryo dormancy and coat dormancy: The components of physiological dormancy
Induction, maintenance and release of physiological dormancy by plant hormones and environmental signals
Variation of the seed dormancy trait in Arabidopsis and Nicotiana
Hyperlink to Seed Dormancy Webpage 2
Phylogenetic table: Seed dormancy classification with examples
Seed Dormancy - Related topics on other webpages
Seed after-ripening:
Dormancy release and promotion of germination
Ecophysiology of dormancy: Response to the environment and dormancy cycling in the seedbank
|
|
|
 |
What is seed dormancy and how is it related to germination? -
Definition of seed dormancy
Seed dormancy could be considered simply as a block to the completion of germination of an intact viable seed under favourable conditions. In our Tansley review 'Seed dormancy and the control of germination' (Finch-Savage and Leubner-Metzger, 2006) we present an integrated view across the evolution, molecular genetics, physiology, biochemistry, modelling and ecophysiology of the control of seed germination by dormancy in an attempt to draw together these linked, but often separate disciplines.
Definition of seed dormancy: A more sophisticated and experimentally useful definition of dormancy has been proposed by Baskin and Baskin (2004).
A dormant seed does not have the capacity to germinate in a specified period of time under any combination of normal physical environmental factors that are otherwise favourable for its germination, i.e. after the seed becomes non-dormant. A completely non-dormant seed has the capacity to germinate over the widest range of normal physical environmental factors possible for the genotype.
Discussion and opinion: Dormancy is a block to germination has evolved differently across species through adaptation to the prevailing environment so that germination occurs when conditions for establishing a new plant generation are likely to be suitable (Finch-Savage and Leubner-Metzger, 2006 and references therein). A diverse range of dormancy mechanisms (blocks) has evolved in keeping with the diversity of climates and habitats in which they operate.
Definitions of dormancy are difficult because dormancy can only be measured by the absence of germination. We can observe completion of germination of a single seed as an all-or-nothing event, whereas dormancy of a single seed can have any value between all (maximum dormancy) and nothing (non-dormancy). Dormancy should not just be associated with the absence of germination, rather it is a characteristic of the seed that determines the conditions required for germination. When dormancy is considered in this way, any environmental cue that alters the conditions required for germination is by definition altering dormancy. Also by extension, when the seed no longer requires specific environmental cues it is non-dormant. Dormancy is a seed characteristic which defines the conditions required for germination and therefore any cue that widens the environmental requirements for germination should be regarded as a dormancy release factor. A wide range of factors can therefore alter (physiological) seed dormancy, e.g. temperature, light, nitrate or naturally occurring chemical signals (ABA and four other terpenes) in leachate from litter that covers the seeds in their habitat. However, there is an important distinction in the seeds response to these factors. 1) There are factors that are related to slow seasonal change. These factors (e.g. temperature) are integrated over time to alter the depth of dormancy, and the sensitivity to other factors (e.g. light). 2) There are other factors that indicate in a more immediate way that conditions are suitable for germination (e.g. light), which could be considered to terminate dormancy and therefore induce germination. Each of these factors therefore remove successive blocks to germination, but this process usually needs to be carried out in a set order for it to work, i.e. in the process described light must come last to be effective. In summary, seed dormancy is an innate seed property, which defines the environmental conditions that must be met before the seed can germinate. The intrinsic molecular mechanisms that determine dormancy have an embryo and/or a coat component. However, dormancy as a “whole seed”-characteristic controls germination and a classification system for seed dormancy has been proposed. See the 'seed dormancy webpage II ' for a phylogenetic table on the dormancy classification.
Primary versus secondary seed dormancy: Freshly harvested mature water-permeable dormant seeds are said to have primary dormancy, which has been induced with the involvement of abscisic acid (ABA) during seed maturation on the mother plant (Finch-Savage and Leubner-Metzger, 2006 and references therein). Subsequent dormancy release “in the field”, following dispersal, may involve the same factors that are commonly used “in the lab”: either after-ripening in the relatively dry state, or dormancy-release treatments in the imbibed state. These imbibed seed treatments include chilling (cold stratification), warm stratification, light, gibberellins (GA) and other hormones (Kucera et al. 2005 and references therein), smoke substances like butenolide and compounds like nitric oxide. In contrast to primary dormancy, secondary dormancy can be induced in seeds with non-deep physiological dormancy after seed dispersal, and is often associated with annual dormancy cycles in the seed bank.
|
 |
A classification system for seed dormancy: The 'whole-seed view'
Marianna G. Nikolaeva devised a dormancy classification system reflecting that dormancy is determined by both morphological and physiological properties of the seed. Based on this scheme, C. Baskin and J. Baskin (1998; 2004) have proposed a comprehensive classification system which includes five classes of seed dormancy. The system is hierarchical with these five classes further divided into levels and types Baskin and Baskin (2004). See the 'seed dormancy webpage II ' for a phylogenetic table on the dormancy classification and examples for the different dormancy classes.
(1) Physiological dormancy (PD)
PD
(dormancy class A according to Baskin and Baskin, 2004) is the most abundant form and is found in seeds of gymnosperms and all major angiosperm clades. It is the most prevalent dormancy form in temperate seed banks and the most abundant dormancy class “in the field”. PD is also the major form of dormancy in most seed model species “in the lab”, including Arabidopsis thaliana, Helianthus annuus, Lactuca sativa, Lycopersicon esculentum, Nicotiana spp., Avena fatua, and several cereals. The molecular mechanisms of PD are the focus of the Tansley review by Finch-Savage and Leubner-Metzger (2006). PD has three levels: deep, intermediate and non-deep.
PD deep
Embryos excised from PD-deep seeds either don’t grow or will produce abnormal seedlings. GA treatment does not break their dormancy. Ca. 3-4 months of cold (subtype a) or warm (subtype b) stratification are required before germination can take place. Examples: Acer platanoides (PD deep subtype a) (Aceraceae; Finch-Savage et al. 1998); Leptecophylla tameiameiae (PD deep subtype b) (Ericaceae; Baskin et al. 2005).
PD intermediate
Embryos excised from PD-intermediate seeds produce normal seedlings. GA promotes germination in some (but not all) species. Seeds require 2-3 months of cold stratification. Dry storage (after-ripening) can shorten the cold stratification period. Example: Acer pseudoplatanus (PD intermediate) (Aceraceae; Finch-Savage et al. 1998).
PD non-deep
The great majority of seeds have non-deep PD. Embryos excised from these seeds produce normal seedlings. GA treatment can break this dormancy and depending on species dormancy can also be broken by scarification, after-ripening in dry storage, and cold (0-10 °C) or warm (>15 °C) stratification. Based on patterns of change in physiological responses to temperature five types of non-deep PD can be distinguished (Baskin and Baskin 2004). Most seeds belong to type 1 or 2, in which the temperature range at which seed germination can occur increases gradually during the progression of non-deep dormancy release from low to higher (type 1, e.g. Arabidopsis thaliana) or from high to lower temperature (type 2, e.g. Helianthus annuus). In addition, the sensitivity of the seeds to light and GA increases as non-deep PD is progressively released. The molecular mechanisms of Arabidopsis seed dormancy cycling have been investigated by transcriptome analyses (Cadman et al. 2006). A model for the cycling of PD non-deep seeds is presented in the section below: "Induction, maintenance and release of physiological dormancy by plant hormones and environmental signals".
Five types of non-deep Physiological seed dormancy (PD) according to Baskin and Baskin (2004)
|
PD non-deep Type 1
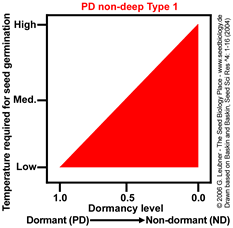
Temperature range for germination increases during dormancy release as a continuum from
low to high.
Example: Arabidopsis thaliana
|
PD non-deep Type 2
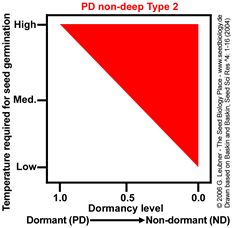
Temperature range for germination increases during dormancy release as a continuum from
high to low.
Example: Helianthus annuus
|
PD non-deep Type 3
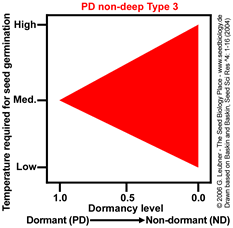
Temperature range for germination increases during dormancy release as a continuum from medium to high and low.
Example: Aster ptarmacoides
|
PD non-deep Type 4 and Type 5
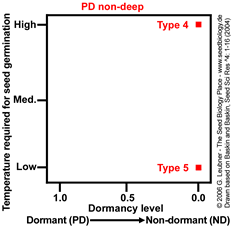
No temperature and dormancy continuum, non-dormant seeds germinate only at:
- high temperature
(Type 4).
Example: Callicarpa americana
- low temperature
(Type 5).
Example: Gentianella quinquefolia
|
 |
 |
(2) Morphological dormancy (MD)
MD (dormancy class B according to Baskin and Baskin, 2004) is evident in seeds with embryos that are underdeveloped (in terms of size), but differentiated (e.g. into cotyledons and hypocotyl-radical). These embryos are not (physiologically) dormant, but simply need time to grow and germinate. This group does not include seeds with undifferentiated embryos. Example: Apium graveolens (Apiaceae).
(3) Morphophysiological dormancy (MPD)
MPD
(dormancy class C according to Baskin and Baskin, 2004) is also evident in seeds with underdeveloped (in terms of size) embryos, but in addition they have a physiological component to their dormancy. These seeds therefore require a dormancy-breaking treatment, e.g. a defined combination of warm and/or cold stratification which in some cases can be replaced by GA application. In MPD-seeds embryo growth/emergence requires a considerably longer period of time than in MD-seeds. Seeds with undifferentiated embryos like the Orchidaceae also have a morphological and a physiological component of dormancy, but they are not considered in this classification scheme (Baskin and Baskin 2004). Examples for MPD: Trollius (Ranunculaceae), Fraxinus excelsior (Oleaceae). There are eight known levels of MPD, based on the protocol for seed dormancy break and germination (see table below).
Type of MPD =
Morphophysiological
dormancy
|
W = warm stratification; C = cold stratification
Temperature requirement |
GA requirement
for
dormancy
release treatment |
Example |
to break dormancy |
at time of embryo growth |
Non-deep simple MPD |
W or C |
W |
yes |
Anemone coronaria |
Intermediate simple MPD |
W + C |
W |
yes |
Aralia mandshuria |
Deep simple MPD |
W + C |
W |
(yes) |
Fraxinus excelsior |
Deep simple epicotyl MPD |
W + C |
W |
(yes) |
Hydrophyllum macrophyllum |
Deep simple double MPD |
C + W + C |
W |
? |
Smilacina racemosa |
Non-deep complex MPD |
C |
C |
yes |
Erythronium albidum |
Intermediate complex MPD |
C |
C |
yes |
Trollius spp. |
Deep complex MPD |
C |
C |
no |
Delphinium tricorne |
 |
 |
 |
 |
 |
(4) Physical dormancy (PY)
PY
(dormancy class D according to Baskin and Baskin, 2004) is caused by one or more water-impermeable layers of palisade cells in the seed or fruit coat (Baskin et al. 2000, Baskin, 2003, Baskin and Baskin 2004). In seeds PY-seeds, prevention
of water uptake develops during maturation drying and the covering layer(s) control water movement (often associated with hardseededness). Seeds will remain dormant until some factor(s) render the covering layer(s) permeable to water. In nature, these factors include high temperatures, widely fluctuating temperatures, fire, drying, freezing/thawing and passage through the digestive tracts of animals. In seed technology, mechanical or chemical scarification can break PY dormancy. Once PY is broken, i.e. the seed or fruit coat becomes permeable to water, the seeds can germinate over a wide range of ambient conditions. Unlike PD-seeds, which may re-enter (secondary) dormancy after primary dormancy is broken, once the coat of PY-seeds becomes permeable it generally cannot revert to complete impermeability. Thus, the timing of dormancy break in nature is a more critical event in the life cycle of plants with PY, than it is in those with PD. The mechanism for PY-break must therefore be fine-tuned to the environment.
Specialized structures for water-impermeability
PY is associated with the main mechanical covering layer(s) of the seed and/or fruit coat. In most hardseeded species a palisade of radially elongated cells prevents water uptake. This palisade layer(s) prevent water uptake by their physical arrangement as a tissue and the chemical coatings/impregnates of the cells (heavy ligninfication, suberin-cutin matrix, waxes). In several cases specialized structures are associated with the control of water-impermeablility (Baskin et al. 2000, Baskin, 2003).
The figure on the right shows the testa (seed coat) structure of a typical legume seed (Fabaceae). Water-impermeability of PY-dormant legume seeds is due to a palisade layer of Malphighian cells in the exotesta. The the lens (strophiole, not shown) is the spezialized structure localized in the hilum region that controls water-impermeability. |
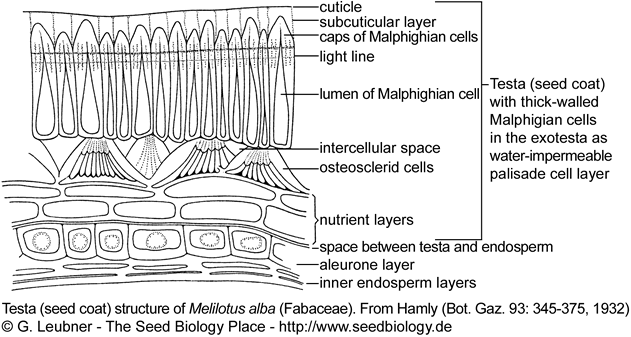 |
In PY-dormant Fabaceae species (Rosids) the lens (strophiole) is the spezialized structure localized in the hilum region that controls water-impermeability. The hardseededness and water-impermeability of many legume seeds is due to a palisade epidermal layer of thick-walled Malpighian cells in the outer testa (exotesta). PY-dormancy of legume seeds is released if the lens is disrupted and a 'water-gap' allows water uptake. In most legume seeds stress, e.g. by heating, causes disruption (pulling apart) of the relatively thin-walled cells of the lens. In some legume species 'pop-up lenses' are evident. There is strong evidence that the lens is the weak point in the palisade layer of PY-dormant Fabaceae seeds. It is however not the only place of water entry: The lens functions as an environmental 'signal detector', it may act as regulator of the rate of water entry into the seed, thereby affecting seed and seedling vigor.
Examples for PY-dormant Fabaceae seeds with lens are Melilotus and Trigonella species.
In PY-dormant Ancardiaceae species (Rosids), e.g. Rhus spp., the endocarp consists of three water-impermeable palisade layers (macrosclereids, osteosclereis, brachysclereids) and the outer crystalliferous layer. The carpellary micropyle is a discontinuity in the macroslereid layer and the specilized structure that controls water-impermeability. No discontinuity occurs in the osteosclereid and brachysclereid layers, and these layers block water entrance to the carpellary micopyle in dormant seeds. Dormancy release by heating causes formation of a blister near the carpellary micropyle via uplifting of the osteosclereid and brachysclereid layers. During blister formation, a slit is formed in these layers, therby creating an opening for water entrance.
Different types of chalazal plugs/caps are the spezialized structures that control water-impermeability of other seeds, e.g. in PY-dormant species of the Malvales clade (Rosids) families Bixaceae, Cistaceae, and Malvaceae. A suberized 'stopper' formed from the nucellus is the spezialized structure that controls water-impermeability of the Geraniaceae (Rosids). An imbibition lid is the spezialized structure that controls water-impermeability of the Cannaceae (Monocots), the only Monocot family with PY species.
(5) Combinational dormancy (PY+PD)
Combinational dormancy, PY+PD
(dormancy class E according to Baskin and Baskin 2004), is evident in seeds with water-impermeable coats (PY) combined with physiological embryo dormancy (PD non-deep). Within the Ancardiaceae the genus Rhus includes species with PY+PD- dormancy (e.g. Rhus aromatica) and species with only PY-dormancy (e.g. Rhus glabra). Embryos of freshly harvested mature PY+PD-dormant seeds of winter annuals, e.g. Geranium spp. (Geraniaceae, Rosids) and Trifolium spp. (Fabaceae, Rosids), have some conditional dormancy (PD non-deep). This PD is released during after-ripening storage, even of the seed coat remains water impermeable (PY). Embryos of PY+PD-dormant Ceanothus spp. (Rhamnaceae, Rosids) are more deeply dormant (but still PD non-deep). In this case PY is released first and the subsequent water uptake during imbibition is followed by the release of the embryo dormancy (PD non-deep) during a few weeks of cold stratification. Thus, release from PY and PD of PY+PD-dormant seeds seems to be by independend events and the timely order can be species-specific.
Seed dormancy webpage II - Phylogenetic table: Seed dormancy classification with examples
|
 |
Evolution of seed structure and seed dormancy
See the 'evolution webpage' for seed evolution, for the seed phylogenetic tree of Martin (1946) and a figure of die phylogenetic distribution of the dormancy classes taken from our Tansley review (Finch-Savage and Leubner-Metzger, 2006). See the 'seed dormancy webpage II ' for a phylogenetic table on the dormancy classification. A general scheme of the evolutionary connection between seed structure and dormancy class is shown below.
MD and MPD are present in B-type seeds, but can also be typical of some specialized species (for the structural seed types see 'seed evolution webpage'). MD is thought to be the ancestral dormancy type among seed plants and is the most primitive dormancy class. The dispersal of seeds with an underdeveloped embryo that need time to grow might have evolved as an ancient strategy to disperse germination over time. MD and MPD are not only typical for primitive angiosperms, but also for primitive gymnosperms like the Zamiaceae, Ginkgoaceae, Podocarpaceae and Taxaceae. Evolution of larger embryo size in MD seeds resulted in non-dormant (ND) seeds. Concurrently, the gain of physiological dormancy mechanism(s) led from seeds with MD to seeds with MPD, which upon gain in embryo size led to PD seeds.
PD is the most phylogenetically widespread dormancy class. This class of dormancy is distributed over the entire phylogenetic tree from gymnosperms and the basal angiosperms to the higher core eudicot Rosid clade. As ND is also distributed over the entire phylogenetic tree it has been proposed that gain and loss of PD occurred at several times and at several levels of seed evolution (see figures on the 'seed evolution webpage').
The most phylogenetically restricted and derived dormancy classes are PY and PY+PD. The occurrence of an impermeable seed or fruit coat combined with a ND embryo (PY) or a PD embryo (PY+PD) is probably the adaptation to specialised life strategies or habitats. PY and PY+PD are the only dormancy classes not found in gymnosperms, and PY is only evident in one Monocot family (Cannaceae).
A conceptual model for the evolution of PY and PY+PD within the Ancardiaceae has been proposed (Baskin et al. 2000). Within this family the genus Rhus includes species with PY+PD- dormancy (e.g. Rhus aromatica) and species with only PY-dormancy (e.g. Rhus glabra). Anatomical evidence for PY of extinct Rhus spp. suggests that PY had evolved in the Ancardiaceae by 43 Ma in the Middle Eocene. The model begins in pre-Eocene times with an ancestral species that has large, non-dormant (ND), recalcitrant seeds. By the middle Eocene, a derived species with relatively small, orthodox seeds and a water-impermeable covering layer had evolved, i.e. a PY-dormant seed evolved. By the Oligocene, PD had been added to the seed dormancy mechanism, i.e. a PY-dormant seed evolved. Climatic drying (Eocene), followed by climatic cooling (Eocene-Oligocene transition), are proposed as primary selective agents in the development of PY. An evolutionary connection between PY and recalcitrance is suggested by the relatively high concentration of these two states in the Rosid clade. Phylogenetic data and fossil evidence seem to support the PY ---> PY+PD evolutionary sequence in Ancardiaceae, which also may have occured in Fabaceae.
|
 |
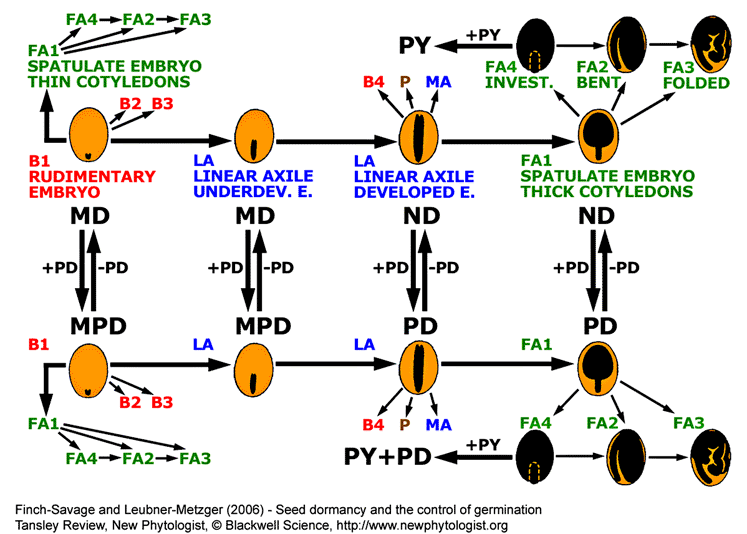
Summary of general trends in angiosperm seed evolution with respect to seed type and dormancy class.
Seeds with small embryo and abundant endosperm (B1 seed type) are primitive and evolution shows a general trend (indicated by arrows) towards mature seeds with little or no endosperm in which the embryo occupies most of the seed (through LA to FA seed types). Gain or loss of PD to each of these seed types (B1, LA and FA1) alters the dormancy class as indicated. Similar changes can also occur in the other seed types. Addition of PY to FA4-type ND or PD seeds is indicated as a side branch occurring in specialized species. This addition can be reversed and PY can also be added to other seed types (not shown). Seed types and dormancy classes are described in the figure above. See the 'seed dormancy webpage II ' for a phylogenetic table on the dormancy classification.
This diagram is based on information given in figures 12.21 and 12.22 of Baskin and Baskin (1998).
Figure published in the
Tansley review "Seed dormancy and the control of germination" by Finch-Savage WE and Leubner-Metzger G (New Phytologist 171: 2006).
A PDF file of this review is available for download from
either "The Seed Biology Place" (Finch-Savage and Leubner-Metzger, 2006)
or the New Phytologist Trust website at
New Phytologist Trust - Tansley Reviews -
© Blackwell Science -
http://www.blackwell-science.com/
|
 |
|
 |
Embryo dormancy and coat dormancy: The components of physiological dormancy
The hierarchical classification system by Baskin and Baskin (2004) accurately reflects the “whole-seed” ecologists view of the control of germination by dormancy. At the molecular level very little is known about MD, MPD, PY, PY+PD and deep PD. Recent work by Cadman et al. (2006) has provided insight into molecular mechanisms of non-deep PD. Thogether with earlier physiological work (Finch-Savage and Leubner-Metzger, 2006) it is clear that the intrinsic molecular mechanisms determining dormancy can have an embryo and/or a coat component. The terms embryo and coat dormancy are used to distinguish between these two mechanisms, but it should be emphasized that they are not used as part of a classification system for “whole-seeds” sensu Baskin and Baskin (2004).
Embryo dormancy and coat dormancy are components of PD; their sum and interaction determines the degree of “whole-seed” PD. Embryo dormancy is characterized by a block that inhibits extension growth and therefore excised embryos do not grow. Coat dormancy, is characterized by a block that is conferred by the covering layers. Non-dormant embryos excised from coat-dormant seeds will therefore extend and grow. “Coat” is used in a loose sense and can be any embryo covering structure, e.g. testa, endosperm and/or pericarp and terms like testa dormancy and endosperm dormancy can be used to specify these. For example, mechanical resistance from combined testa and endosperm dormancy, which is greater than the embryo growth potential opposing it, appears to be the cause of non-deep PD in seed model systems like A. thaliana and Solanaceae species. When embryo and coat dormancy are considered as components of non-deep PD the influence of ABA and GA on dormancy and the control of germination can be summarised as follows:
Embryo dormancy
Embryo dormancy (embryo component of non-deep PD): A dormant embryo is characterized by a high ABA/GA ratio, high ABA sensitivity and low GA sensitivity. Embryo dormancy release involves remodelling of hormone biosynthesis and degradation towards a low ABA/GA ratio, a decrease in ABA sensitivity and an increase in GA sensitivity. Thus ABA dominates the embryo dormancy program, and GA the embryo germination program. A non-dormant embryo is characterized by increased growth potential, the ability for cell extension growth and the ability to induce the release of coat dormancy.
Coat dormancy
Coat dormancy (coat component of non-deep PD): The combination of an embryo with low growth potential and mechanical constraint from the seed covering layers can result in dormancy. GA can release this coat dormancy by increasing the embryo growth potential and/or by reducing the mechanical constraint. There are two forms which are distinguished based on whether the covering layer consists of dead (e.g. outer testa) or living (e.g. endosperm) tissue:
(1) Testa dormancy: ABA during seed development determines the subsequent GA requirement for germination since ABA influences the testa characteristics (e.g. thickness) and GA the embryo growth potential.
(2) Endosperm dormancy: Endosperm weakening can be either part of the coat dormancy release or part of the germination program. Since the endosperm is in most cases living tissue it can actively participate in regulating embryo constraint by influencing both the ABA/GA ratio and sensitivity to these hormones. GA acts by increasing the embryo growth potential and by promoting endosperm weakening which is achieved though ABA-independent and ABA-inhibited mechanisms.
|
 |
|
 |
Induction, maintenance and release of physiological dormancy by plant hormones and environmental signals
There is considerable evidence that abscisic acid (ABA) is an important positive regulator of both the induction of dormancy and the maintenance of the dormant state in imbibed seeds following shedding (Kucera et al., 2005, Finch-Savage and Leubner-Metzger, 2006).
- ABA-deficiency during seed development is associated with absence of primary dormancy in the mature seed, whereas over-expression of ABA biosynthesis genes can increase seed ABA content and enhance seed dormancy or delay germination (see the table "Arabidopsis hormone mutants").
- ABA produced by the seed itself during seed development can impose a lasting dormancy, whereas, maternal ABA or ABA application during seed development both fail to induce a lasting seed dormancy, but has other functions.
- In Arabidopsis thaliana, the members of the AtNCED gene family encode enzymes catalyzing the key regulatory step in ABA biosynthesis. ABA synthesis in the embryo and the endosperm both seem to contribute to the induction of seed dormancy.
- High ABA contents are present in the imbibed seeds of the strongly dormant A. thaliana ecotype Cape Verde Island (Cvi) and decrease as dormancy is lost. A recent transcriptome analysis with this ecotype strongly supports the view that increased ABA biosynthesis is associated with the dormant state (Cadman et al. (2006).
- De novo ABA biosynthesis during imbibition of dormant, but not non-dormant, seeds has been demonstrated in the A. thaliana ecotype Cvi, as well as in other species including Nicotiana plumbaginifolia, Helianthus annuus, and Hordeum vulgare (Finch-Savage and Leubner-Metzger, 2006). This de novo ABA biosynthesis has been interpreted as a mechanism for dormancy maintenance.
- Work with the strongly dormant A. thaliana ecotype Cvi shows that dormancy may depend on an intrinsic balance of gibberellin (GA)- and ABA-biosynthesis and catabolism, which will determine the dominance of either of the hormones. While PD release of Cvi seeds occurs effectively by after-ripening, stratification or inhibition of ABA biosynthesis, the addition of GA appears less effective. GA treatment of dormant Cvi seeds caused a transient increase in ABA levels, suggesting that in dormant seeds a feedback mechanism exists that maintains a high ABA/GA ratio. Thus, the net result of the dormant state is characterized by increased ABA biosynthesis and GA degradation. It appears to be the ABA/GA ratio, and not the absolute hormone contents, that controls germination (see figure below).
|
 |
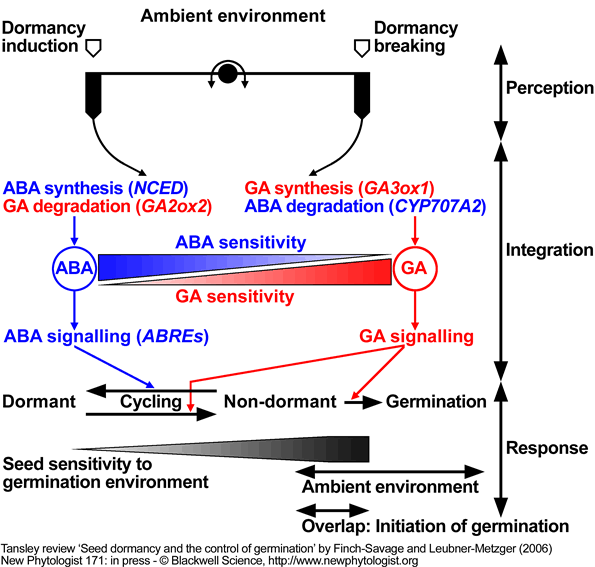 |
 |
Model for the regulation of dormancy and germination by ABA and GA in response to the environment.
According to this model ambient environmental factors (e.g. temperature) affect the ABA/GA balance and the sensitivity to these hormones. ABA synthesis and signalling (GA catabolism) dominates the dormant state, whereas, GA synthesis and signalling (ABA catabolism) dominates the transition to germination. The complex interplay between hormone synthesis, degradation and sensitivities in response to ambient environmental conditions can result in dormancy cycling. Change in the depth of dormancy alters the requirements for germination (sensitivity to the germination environment); when these overlap with changing ambient conditions, germination will proceed to completion.
Model based on work with A. thaliana ecotype Cvi, modified from Cadman et al. (2006). Key target genes are in parenthesis.
Figure published in the
Tansley review "Seed dormancy and the control of germination" by Finch-Savage WE and Leubner-Metzger G (New Phytologist 171: 2006)
A PDF file of this review is available for download from
either "The Seed Biology Place" (Finch-Savage and Leubner-Metzger, 2006)
or the New Phytologist Trust website at
New Phytologist Trust - Tansley Reviews
© Blackwell Science -
http://www.blackwell-science.com/
|
 |
|
 |
Variation of the seed dormancy trait in Arabidopsis and Nicotiana
Arabidopsis thaliana
Non-dormant seeds of Arabidopsis thaliana (Arabidopsis, Brassicaceae) germinate in the presence of water, oxygen, light and a suitable temperature, but dormant Arabidopsis seeds do not germinate at these favorable ambient conditions. Arabidopsis is native to Europe and central Asia. Flowering time and seed dormancy are key traits that determine the timing and lenght of the Arabidopsis natural life cycle (Koornneef et al. 2004). For both traits extensive natural variation is present among the different Arabidopsis accessions, which determine the variation observed in life cycles and the climatic adaptations. In Europe, Arabidopsis flowers most abundantly in spring and early summer, and mature seeds are dispersed from May to July. These seeds exhibit a certain degree of conditional dormancy (PD non-deep), most of them germinate in the fall and survive winter as rosettes and therefore are winter annuals. Under greenhouse conditions these Northern European winter annual accessions flower late, but are very responsive to vernalization (cold treatment), which under natural conditions synchronizes flowering in springtime. In general, most accessions collected from nature in Northern Europe are late flowering are typical winter annuals; Southern populations are of both types, summer and winter annuals.
Seed dormancy is a quantitative trait, determined by several genes with an additional strong impact of the environment (Finch-Savage and Leubner-Metzger, 2006). The analysis of natural alleclic variation at seed dormancy (PD nondeep) loci of Arabidopsis thaliana was performed by exploiting these differences of accessions in the degree of dormancy. The laboratory strains Columbia (Col) and Landsberg erecta (Ler) have low dormancy, whereas the Cape Verde Island (Cvi) and C24 accessions have deep dormancy. Recombinant inbred lines (RILs) / near isogenic lines (NILs) between Cvi and Ler were used in a quantitative trait loci (QTL) approach (Alonso-Blanco et al. 2003) and identified several loci, named "Delay Of Germination 1 to 12" (DOG1 to DOG12), that determine Arabidopsis seed dormancy. DOG1, for which the Cvi allele increased the level of seed dormancy, explains 12% of the variance observed in seed dormancy in these RIL population. The DOG1 gene has been cloned and is a member of a small gene family of unknown molecular function (Bentsink et al. 2006). The functional allelic variation present in Arabidopsis is caused by polymorphisms in the cis-regulatory region of the DOG1 gene resulting in considerable expression differences between DOG1 allels of the accessions analyzed. Alternative splicing leading to DOG1 proteins that differ in the C-terminal domain also appears to be important for dormancy. Further details on Arabidopsis dormancy genes: Website of the group of Wim Soppe (Department of Maarten Koornneef, MPI for Plant Breeding Research, Köln, Germany).
Seed dormancy and germination of Arabidopsis are controlled by phytohormones and an interaction between the growth potential of the radicle and the mechanical resistance of the seed covering layers (Kucera et al. 2005; Müller et al. 2006; Koornneef et al. 2002). Two-step seed germination of Arabidopsis is visible as (1) testa rupture and (2) endosperm rupture. Only the endosperm rupture step is inhibited by ABA, but ABA during seed maturation is involved in determining the degree of Arabidopsis seed dormancy. Germination of dormant Arabidopsis seeds is blocked prior to testa rupture, and GA is required for its induction. Seed dormancy of mutants affected in ABA and GA levels and sensitivity is altered: ABA-deficiency (aba1 mutant) and ABA-overproduction (cyp707a mutants) lead to non-dormancy and increased dormancy, respectively. The ABA-insensitive mutant abi3 is non-dormant. GA-deficiency (ga1 mutant) leads to increased dormancy. Mutations in DELLA-type repressors mediating of GA-responses in seeds lead either to enhanced (gain-of-function) or to reduced (loss-of-function) dormancy.
Control of germination by the endosperm - Arabidopsis seed dormancy is primarily determined by the endosperm: A publication by Bethke et al. (2007) demonstrates that the Arabidopsis endosperm layer is sufficient and necessary for seed dormancy. Embryos from dormant seeds had a lesser growth potential than those from nondormant seeds. However, the endosperm layer was found to be the primary determinant of seed dormancy. Arabidopsis endosperm (aleurone) cells responded to nitric oxide (NO), GA, and ABA, with NO being upstream of GA in a signaling pathway that leads to vacuolation (GA-induced, ABA-inhibited) of protein storage vacuoles. Dormancy release and the GA signal lead to Arabidopsis endosperm rupture which involves these microscopic changes that are typical for endosperm weakening (Bethke et al., 2007; Müller et al., 2006).
Control of germination by the seed coat - Arabidopsis testa mutants: Completion of germination in seeds with PD-type coat dormancy requires that either the embryo growth potential increases to overcome the mechanical constraint, and/or the mechanical constraint associated with the seed covering layer(s) is reduced (Finch-Savage and Leubner-Metzger, 2006). Two forms of mechanical constraints and release mechanisms can be distinguished: (1) living seed covering layers where a regulated tissue weakening occurs prior to germination and the tissue itself can produce enzymes for this process, e.g. of endosperm or inner testa; (2) mostly dead seed covering layers where predetermined breaking points facilitate tissue rips prior to germination, e.g. outer testa (this section) or pericarp. The testa is maternal tissue and the reduced seed dormancy phenotype is inherited maternally. A series of Arabidopsis testa mutants show reduced dormancy that is caused by alterations of the testa characteristics (Debeaujon and Koornneef 2000; Koornneef et al. 2002). Examples are tt (transparent testa), ttg (transparent testa glabra), and ats (aberrant testa shape). The testa mutants highlight the importance of the testa structure as a constraint to radicle emergence. The GA requirement for Arabidopsis seed germination is determined by testa characteristics, embryonic growth potential and by embryonic ABA.
Arabidopsis seed dormancy genes: These genes are represented by Arabidopsis mutants affected in seed dormancy and include hormone-related genes (table "Arabidopsis hormone mutants"), and other genes (Koornneef et al. 2002; Bentsink et al. 2006) that affect dormancy via the testa characteristics (testa mutants; see paragraph above) or by different mechanisms, e.g. fus3 (fusca3), lec1 and lec2 (leafy cotyledons), dag1 and dag2 (DOF affecting germination, DOF-type transcription factors), rdo1 to rdo4 (reduced dormancy), cts (comatose), and DOG1 (delay of germination).
Nicotiana spp. - tobacco
Nicotiana spp. seed dormancy and ABA (review and references: Leubner-Metzger 2003): The onset of dormancy in Nicotiana tabacum is correlated with a peak in ABA content at approximately 15-20 days after pollination (DAP), and a rapid decline in ABA content follows during further seed maturation. Tobacco seeds harvested after DAP 25 are dark-brown, the embryos are mature and have white cotyledons, the products of maturation-specific genes have accumulated, dormancy has been established, desiccation tolerance has been acquired, and the moisture content is low. Seed dormancy is not established in transgenic tobacco expressing an anti-ABA antibody that causes deficiency in free ABA, resulting in precocious germination, embryos with green cotyledons, reduced accumulation of storage proteins, and desiccation intolerance. Nicotiana species differ in the degree of seed dormancy (PD non-deep): N. tabacum and N. sylvestris have a low level of dormancy (exception: photodormancy of N. tabacum cv. Havana 425, see paragraph below), while N. plumbaginifolia (Grappin et al. 2000) has a somewhat higher degree of dormancy. The high degree of seed dormancy of the post-fire annual tobacco, Nicotiana attenuata, can be induced by ABA and terpenes and smoke-exposure, light and GA are required for dormancy release (Krock et al. 2002; Schwachtje and Baldwin 2003).
The wilty mutants aba1 and aba2 of Nicotiana plumbaginifolia have reduced ABA contents and exhibit precocious germination and reduced primary dormancy (review: Leubner-Metzger 2003). ABA-deficiency of the aba2 mutant is due to a mutation in the ABA2 gene, encoding zeaxanthin epoxidase, a key step in ABA biosynthesis. Antisense- and sense-ABA2 transformation of N. plumbaginifolia resulted in decreased and increased ABA biosynthesis and seed dormancy, respectively. Experiments suggested that, as in Arabidopsis and tomato, also in Nicotiana species only ABA produced by the seed itself, but not maternal ABA, is necessary to impose a lasting dormancy. Tobacco dormancy can be released during after-ripening, i.e. a period of dry storage of freshly harvested, mature seeds. A further decline in ABA content, decreased sensitivity to ABA and increased sensitivity to GA are involved in the after-ripening-mediated transition from the dormant to the non-dormant state of many species. The work of Grappin et al. (2000) demonstrated this for N. plumbaginifolia and showed in addition de novo ABA biosynthesis in imbibed fresh (dormant), but not after-ripened (non-dormant) seeds. Promotion of ABA-delayed seed germination of N. plumbaginifolia by light or GA involves stimulation of ABA degradation and inhibition of ABA synthesis
Tobacco photodormancy: Light is required for at least two aspects of tobacco seed germination (review: Leubner-Metzger 2003). First, in photodormant tobacco seeds germination in darkness is blocked at a step before testa rupture and neither neither testa nor endosperm rupture occur even after several weeks of dark-imbibition. Brief treatment of imbibed photodormant seeds with red light activates the phytochrome signal transduction pathway resulting in the release of photodormancy and the promotion of germination. Genetic and physiological experiments suggest that tobacco photodormancy is mainly under maternal control. Gibberellin (GA) can substitute for the red-light trigger needed to release photodormancy and to induce testa rupture and subsequent endosperm rupture of tobacco seeds imbibed in the dark. Red light has been shown to up-regulate the biosynthesis of bioactive GA in germinating seeds of lettuce and Arabidopsis. Fresh tobacco seeds are photodormant and after-ripening contributes to the release of photodormancy (see webpage "after-ripening"). This effect varies greatly for different seed batches as reported for several tobacco cultivars. The GA requirements for photodormancy release of fresh and completely photodormant after-ripened seed batches are equal. Non-photodormant tobacco seeds have lost the GA requirement for dark-germination, which could be due to increased GA sensitivity and/or increased endogenous GA levels. Light is also required for a second aspect of tobacco seed germination, it promotes the speed of endosperm rupture of non-photodormant tobacco seeds. GA is also not only involved in inducing dark-germination of photodormant tobacco seeds, it also promotes ABA-delayed endosperm rupture of dark-imbibed non-photodormant seeds, and osmoticum-delayed testa and endosperm rupture of light-imbibed seeds. Finally, endogenous ethylene and brassinosteroids also promote the germination of non-photodormant tobacco seeds and counteract the inhibitory effects of ABA on endosperm rupture, but ethylene and brassinosteroids do not release tobacco photodormancy.
|
 |
|
|
|
 |
|
|
|
 |
|
 |
| |
|
|
|


 |